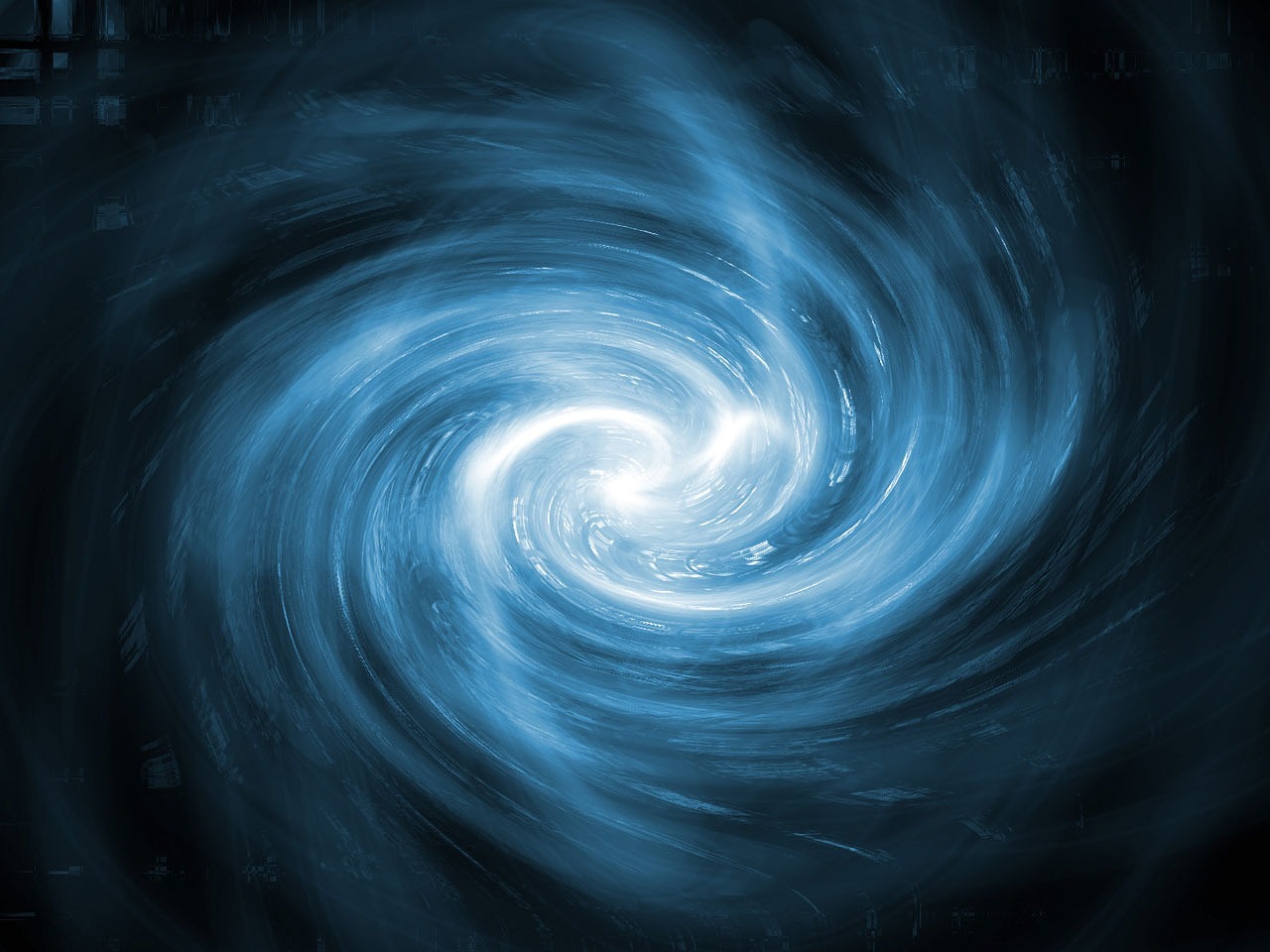
According to the fundamental laws of quantum mechanics, every particle has also an undulatory nature. The characteristic wavelength associated with an elementary particle depends on its mass and speed. Very fast electrons, albeit very light objects, have a very short associated wavelength which makes them ideal for high-resolution microscopy experiments. Using electrons as imaging particles allows to resolve individual atoms in materials.
In conventional imaging with light, one measures how much light of different color hits a photographic film yielding a two-dimensional image of an object. To obtain 3-D information on an object, holography is used instead. In conventional holography, two light beams are used to retrieve not only the intensity and color information but also the phase information on the light scattered by an object. The phase specifies the position of a point within the wave cycle of the photon and correlates to depth of information, meaning that recording the phase of light scattered by an object can retrieve its full 3D shape, which cannot be obtained with a simple photograph. This is the basis of optical holography, popularized by fancy holograms in sci-fi movies like Star Wars. In practical terms, the two beams are spatially separated; one is used as a reference and one is used for imaging.
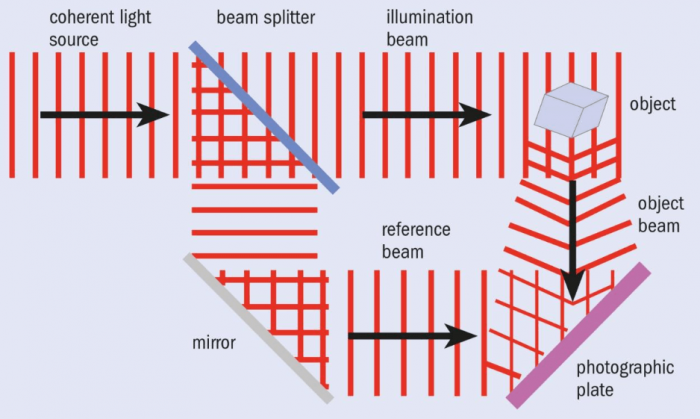
Fig. 1. Schematic representation of optical holography for 3D object reconstructions (at the moment just a suggestion).
Because the wavelength associated to an electron is much shorter than the typical wavelength of a photon, electron holography can resolve much smaller three-dimensional objects (see Fig. 1), providing direct imaging of each individual atoms in a nanoparticle. Both techniques, electron and light holography, yield static 3D images of an object.
One current challenge is to develop further these methods to provide both spatial and temporal resolution, thus allowing to retrieve amplitude and phase information of fast-changing objects. In the laboratory for Ultrafast Microscopy and Electron Scattering at the EPFL in Switzerland, researchers developed a new holographic method capable of resolving fine details down to the nanometer spatial scale with attosecond (10-18 sec) temporal resolution. To demonstrate this achievement, they filmed the propagation of light in a nano-sized antenna.
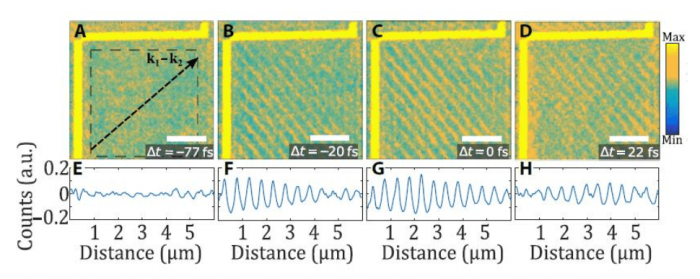
Fig. 2. Microscopic holograms of propagating light field at different temporal instants following the photo-excitation. Scale bars, 2 mm. The nanoscale field emitted from the vertical slit propagates from left to right. Correspondingly, the interference pattern moves from the bottom-left to the top-right corner.
The method itself is an exotic implementation of electron holography. Instead of using two-spatially separated streams of electrons for the reference and the imaging beam, they exploited the ability of light to split an electron wavefunction into different energy levels. The electrons of different energies served as both reference and imaging beam, differently to conventional holography in which imaging and reference beams are separated in space. The advantage of this method is that light can be used to control the splitting of the electron beam dynamically, at the attosecond time scale.
Another benefit of this technique is that while light splits in energy the wavefunction of an electron, it can imprint on it controllable quantum information which becomes encoded into the holographic pattern that is imaged. This offers new perspectives for quantum computation, as in principle it gives the possibility to store multi-dimensional quantum information in a hologram that can be manipulated using light pulses.
At the core of this new method lies the ability of light pulses to manipulate the wavefunction of individual electrons. Such an ability can also be further exploited to literally twist them, obtaining a so-called vortex electron beam.
Vortices are very common in nature, from tornadoes, cyclones, and galaxies all the way to elementary particles, which can acquire a vortex-like nature when they spiral around a fixed axis like the tip of a corkscrew. When particles move like this, they form what we call “vortex beams.” These beams are very interesting because they imply that the particle has a well-defined orbital angular momentum, which describes the rotation of a particle around a fixed point.
Because the intrinsic characteristics of the elementary particle have been modified, its interaction with other objects will also change. This means that vortex beams can provide us new radiation/matter interactions. Recently, it has been shown for example that vortex beams have an enhanced sensitivity to magnetic fields. Another promising possibility is that such beams can enable new absorption channels for the interaction between radiation and tissue in medical treatments (e.g. radiotherapy). Furthermore, twisting an elementary particle in a controlled fashion could be also useful to reveal its inner structure. Insights on the neutrons inner structure have been revealed by a similar method for example.
Commonly, the modulation of a particle’s wave function is done using a “passive phase mask,” which can be thought of as a standing obstacle in the water, modulating the waves passing by. An appropriate phase mask, like a hole in the sink, for example, can result in the formation of a whirlpool. So far, physicists have been using the passive phase mask method to make vortex beams of electrons and neutrons.
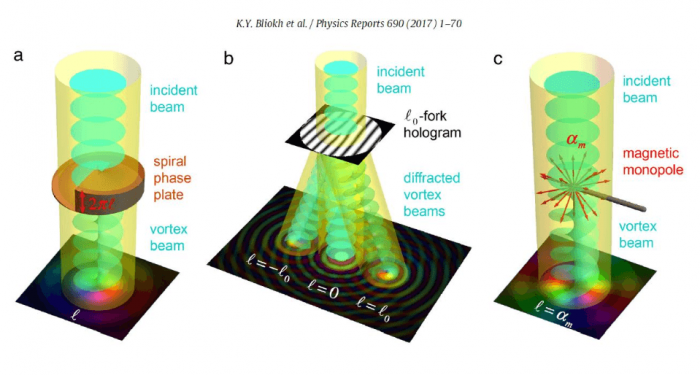
Fig. 3. A schematic representation of the static generation of vortex electron beams using a spiral phase plate (a), a fork hologram (b), and a magnetic monopole (c). Reproduced from Bliokh et al., Phys. Rep. 690 1-70 (2017).
But now, scientists from the lab of Fabrizio Carbone at EPFL demonstrated for the first time that it is possible to use light to dynamically twist an individual electron’s wave function. The researchers were able to generate an ultrashort vortex electron beam and actively switch its vorticity on the attosecond (10-18 seconds) timescale.
To do this, they used very strong localized light fields to generate the necessary phase mask. Firing ultrashort electron pulses, synchronized to the maximum strength of the light fields, resulted in their wavefunction to be twisted (see Fig 4).
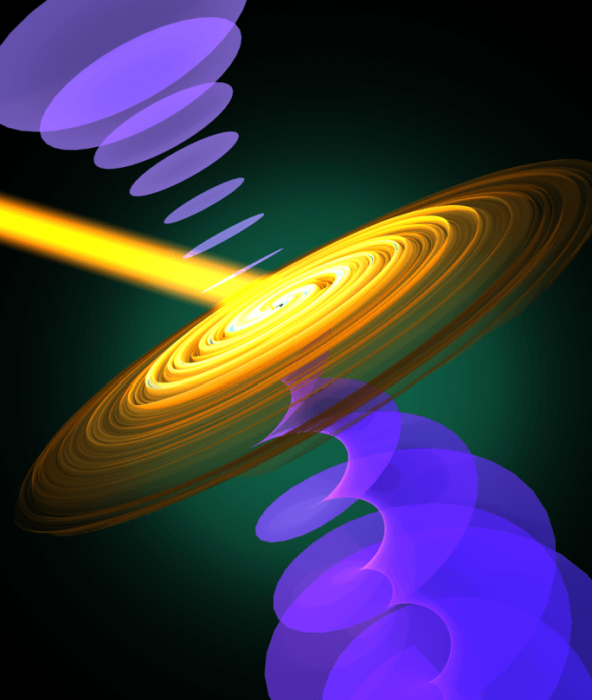
Fig. 4. A schematic representation of the OAM transfer to electrons: the interaction between a pulsed electron plane wave synchronized with a chiral plasmonic field creates an OAM-carrying vortex electron wave packet. Credit: Ella Maru Studio.
There are many practical applications from these experiments. Ultrafast vortex electron beams can be used to encode and manipulate quantum information, and the electrons’ orbital angular momentum can be transferred to the spins of magnetic materials to control the topological charge in new devices for data storage. But, even more intriguingly, using light to dynamically “twist” matter waves offers a new perspective in shaping protons or ion beams such as those used in medical therapy, possibly enabling new radiation-matter interaction mechanisms that can be very useful for selective tissue ablation techniques.