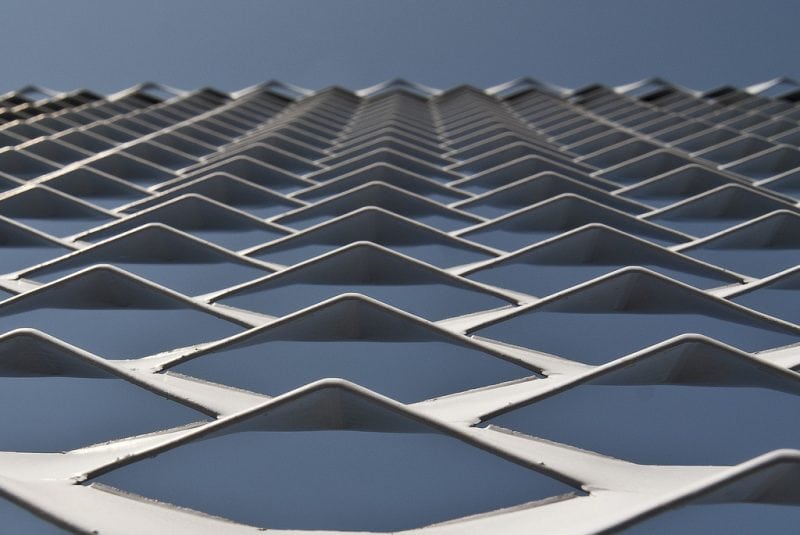
Aluminum is an abundant metal in the Earth’s crust and has become widely used in a large diversity of applications also due to its desirable physical-chemical properties. The usage of aluminum has increased enormously in recent years, and in the foreseeable future the demand for aluminum products is expected to increase even more, at a rate of 4% per year in the next 5 years, and this figure will certainly increase after that.
This increase is due not only to the areas where aluminum is currently employed, such as transportation, manufacturing, construction, electrical applications, and consumer goods, but also in areas that are expected to emerge as the result of new technological developments, as in for example fuel-cell powered vehicles.
More advanced technological applications are accompanied by higher demands in manufacturing. These demands are constantly increasing due to the increased sophistication of products and of their more complex manufacturing processes. This is because higher sophistication requires better control of the physical-chemical properties and the margins for error become smaller. Aluminum products are no exception to this and the process of anodization (controlled oxidation) of aluminum is a widely used but technologically demanding process.
Anodization is an important process for providing protection and functionality to the material. In recent years new applications for this metal have pushed the boundaries of the anodization processes and this led to the need of knowledge on the reactions between oxygen and aluminum at a fundamental level — at the atomic and electronic scales. Such knowledge is necessary for understanding processes that are the result of atomic-scale phenomena and that occur in very short time and length scales but that still determine how things develop for example in a manufacturing process.
In recent years, quantum mechanical studies of real systems have become possible due to the increase in the computational power of supercomputers, increased efficiency of new parallelization algorithms and the development of more robust algorithms to handle the complicated equations that describe the world of quantum mechanics. In this sense, supercomputers are becoming indispensable tools for the study of phenomena such as oxidation, which is difficult to study experimentally due to the very short time scales in which occurs and due to its highly dynamical nature.
The interplay between kinetically and thermodynamically controlled processes adds difficulties to experimental measurements and not always it is possible to obtain thermodynamic information of quality due to kinetic effects. In this sense, the results of quantum mechanical modeling these processes gives information that is not within reach of experiments. The challenges are then to set up models that can be used in quantum mechanical modeling but that can represent the systems. In practice, this means that the models have to be large enough in order to be valid representations of the macroscopic world but at the same time have to be small enough to be handled by the available supercomputers because these computations imply very large computational loads.
Using quantum mechanical modelling within the formalism of density functional theory (DFT) and periodic boundary conditions, C. Lousada and P. Korzhavyi from The Royal Institute of Technology – KTH, Sweden, studied the mechanisms of the first stages of the oxidation of an aluminum surface, scanning through a variety of possible atomic structures and mechanisms. The researchers used static nucleus DFT calculations followed by DFT-based molecular dynamics to confirm that the finds are valid at finite temperatures and are the correct mechanisms for the growth of an oxide in laboratory conditions.
During the oxidation of a pure metallic surface exposed to gas-phase O2, oxygen reacts in a random way with the material. Because of this, the existing picture of the oxidation process is that with increasing number of incoming O2 there is a disposition of O-atoms at the surface that is fairly homogeneous and this would correspond to the thermodynamically favored structure because in this case the stresses induced by the O-atoms and the overall dipole moments are more homogeneously distributed at the surface. However, these researchers found that for that aluminum surface there are two different main mechanisms that dominate the growth of the oxide.
The prevalence of each of the mechanisms depends on the coverage of oxygen atoms and the prevalent mechanism changes with the growth of the oxide even for very low coverages of oxygen. Up to a certain coverage of O-atoms at the surface, the growth of oxide proceeds through the formation of aggregates of O-atoms as low symmetry structures with the form clusters or islands. With increasing coverage of O-atoms these islands start to form rows of aluminum oxide and after a certain coverage has been reached, the oxide starts to be formed in a more homogeneous way and gives rise to less defective and higher symmetry structures.
However, this change in growth mode implies large surface reconstructions and adds a significant amount of stress to the surface. The result is that the oxide formed will be defective and rough. This will have repercussions in the quality of the obtained surface films where roughness and defects are undesirable in the context of anodization.
These findings are described in the article entitled First stages of oxide growth on Al(1 1 0) and core-level shifts from density functional theory calculations, recently published in the journal Applied Surface Science. This work was conducted by Cláudio M. Lousada and Pavel A. Korzhavyi from the KTH Royal Institute of Technology.