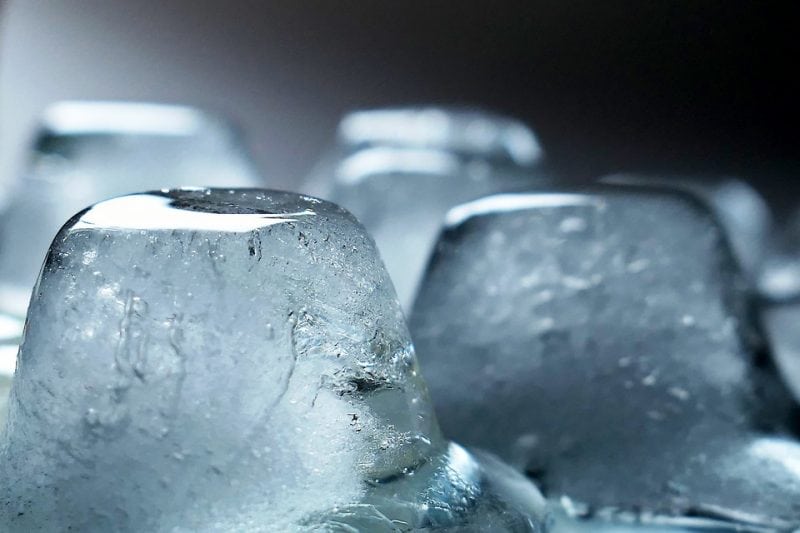
Common ice (Ih) floats on top of water because it is less dense than liquid water. The hollow structure of ice can accommodate small gas molecules as “guests” in its cavities under the right temperature and pressure conditions. These crystalline solids in which guests are accommodated by water (hosts) are called gas hydrates or clathrate hydrates. Several lattice structures of gas hydrates form at different temperature and pressure conditions and guest compositions. Both pure (single-component) and mixed (multi-component) gas hydrates can form.
The concentrations of gas that can be contained inside hydrates as a guest is much higher than the solubility of the same gas in liquid water. For example, 1 m3 of methane hydrate can contain as much as 170 m3 of methane gas at the standard temperature and pressure (STP). In contrast, the solubility of methane in liquid water is of the order of 10-5 in mole fraction at the STP. This high gas content in hydrates can be useful in gas storage, especially for permanent and large-scale gas storage such as carbon dioxide sequestration. On the other hand, gas hydrates are undesirable in oil and natural gas pipelines where its formation can lead to blockage and pipeline damage.
Natural gas hydrate deposits, mainly methane, form the largest unconventional natural gas resources in the world. These natural gas hydrate deposits are not economically viable for development at present, however, history shows that the development of a range of unconventional oil and gas resources has steadily moved the “resource food chain” downward. In fact, recent history revealed that a range of unconventional oil and gas resources, which were once considered uneconomical to develop, eventually became accessible when their time came.
Gas hydrates also have significant implications in the context of climate change. Methane is about 20 times more potent as a greenhouse gas than carbon dioxide, so its uncontrolled release into the atmosphere could be potentially catastrophic. One possible scenario in which natural methane hydrate deposits, if left unattended, could lead to such a catastrophic release of methane gas is through a positive feedback loop. Here, gradually rising global temperatures could lead to unintended dissociation of a small portion of the massive natural methane hydrate deposits. The release of methane gas from said dissociated deposits to the atmosphere would further raise the global temperature through the greenhouse gas effect, which in turn would lead to a little bit more dissociation of the natural methane hydrate deposits, and so on.
Ideally, we wish to be able to extract methane gas from the methane hydrate deposits for use as our fuel and replace it with carbon dioxide hydrates. Carbon dioxide hydrate is thermodynamically more stable than methane hydrate, so methane hydrate in contact with carbon dioxide gas is expected to convert to methane gas and carbon dioxide hydrate if one waits long enough. However, such conversion needs to proceed in a timely manner and in large scales for it to be practical. In short, we wish to be able to accelerate gas hydrate formation when desired and decelerate its formation when undesired.
Nucleation is the first step of any phase transition. Nucleation requires surmounting of an activation energy barrier as described by Arrhenius’ law. A phase transition can only proceed after ubiquitous fluctuations allow the formation of critical nuclei over the activation barrier, the size of which depends on the subcooling of the system. This is intrinsically a stochastic process even for a single-component system. For a multi-component system, the availability of each component and its rate of mass transfer add to the complexity of the stochastic process. This is especially the case where some components have limited availability as is the case for gas hydrate systems.
Nucleation of gas hydrates has been a vastly under-researched area because of its sheer complexity and limited means for its systematic investigations. In particular, nucleation rate, the most basic measure of nucleation, proved difficult to study in the past. Over the last few years, however, a quiet shift has been taking place in a way the nucleation rate of gas hydrates is being investigated [1-4]. Heterogeneous nucleation rates of natural gas hydrates per unit area of guest gas – aqueous interface in the absence of a solid wall were measured for the first time. The recent study also called for a fundamental change in how the heterogeneous nucleation rate should be defined for gas hydrate systems.
According to the new theory, the heterogeneous nucleation rate of gas hydrates should be normalized to the unit area of aqueous-guest gas interface in the absence of a solid wall whereas in the presence of a solid wall it should be normalized to the unit length of the three-phase line where the aqueous phase, the guest gas phase, and the solid wall meet, in marked contrast to the heterogeneous nucleation of single component systems [1]. The two key concepts are: (1) the nucleation rate per unit length of the three-phase line is a unique property for the solid in question, (2) the greater of the total nucleation rate integrated over the whole length of the three-phase line in a system or the total nucleation rate integrated over the whole surface area of the guest gas-aqueous interface in the system determines the overall nucleation rate of the system.
If this new theory can be validated and scaled up, and the nucleation rate of common guest gases are measured and compiled, then it will allow the nucleation probability of gas hydrates in a given system to be calculated with the total aqueous – guest gas interfacial area and the total lengths of the three-phase lines in the system as the input parameters.
These findings are described in the article entitled Nucleation curves of methane hydrate from constant cooling ramp methods, recently published in the journal Fuel. This work is one part of the larger work noted in the references below. This work was conducted by Nobuo Maeda at CSIRO in Australia. Dr. Maeda is currently with the University of Alberta.
References:
- Maeda, N., Nucleation Curves of Model Natural Gas Hydrates on a Quasi-Free Water Droplet. AIChE Journal, 2015. 61(8): p. 2611-2617.
- Maeda, N., Nucleation curves of methane – propane mixed gas hydrates in hydrocarbon oil. Chemical Engineering Science, 2016. 155: p. 1-9.
- Maeda, N., Nucleation curves of methane-propane mixed gas hydrates in the presence of a stainless steel wall. Fluid Phase Equilibria, 2016. 413: p. 142-147.
- Maeda, N., Nucleation curves of methane hydrate from constant cooling ramp methods. Fuel, 2018. 223: p. 286-293.