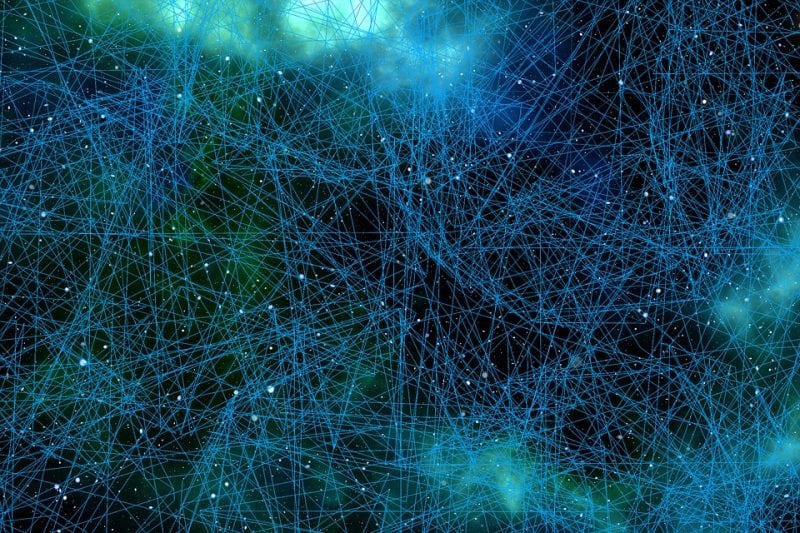
Our brain is one of the most complex biological structures known. It contains billions of nerve cells (neurons) which form a densely interconnected network comprising trillions of connections between these cells.
One of the principal communication forms in this neural network is through electrical signals called spikes or action potentials. Spikes are very short (about a thousandth of a second) and very weak events (~0.1 mV when measured close to the cell which is ten-thousandth of the voltage of a common 1.5 V AA size battery). Fortunately, these brief electrical impulses can be monitored using electrodes (or neural probes) implanted into the brain tissue. Current investigations in the field of neuroscience are largely based on the inspection of the spiking patterns of dozens or even hundreds of individual neurons recorded simultaneously.
Commonly-used neural probes have dimensions comparable to the size of the cell body of neurons (~0.01-0.1 mm, that is, about the diameter of a human hair). First electrodes used to detect the electrical impulses of neurons were thin (~0.05-0.1 mm) insulated wires with the insulation layer removed at the tip of the wire, then placed into the brain tissue. Even with a single electrode, we can monitor the spikes of many nearby neurons, that is, a usual neuronal recording contains the electrical impulses of multiple neurons.
In general, the size (amplitude) and the shape of recorded spikes depend on the distance and the position of the neuron relative to the electrode. For instance, spikes of closer neurons will be larger in size, while the action potentials of brain cells located farther away from the electrode will have a smaller amplitude on the recordings. Since the shape of spikes fired by different neurons may be very similar (especially if the cells are at nearly the same distance from the electrode), separation of the spikes of an individual neuron from the spikes of other neurons is not a trivial task. However, using multiple electrodes located close to each other can aid this separation process.
Let me illustrate this with an example. Imagine that the neurons are birds and the electrode is a microphone which records the singing of these birds (recorded song corresponds here to the recorded spikes). Let’s assume that there are two identical birds sitting at an equal distance from the microphone. In case they are singing the same song with the same volume, then we cannot tell which bird sings at a given moment since the songs will arrive at the same time to our recording device (the sound has to travel the same distance from the two birds to the microphone). However, if we place multiple microphones (for example three) close to each other, then, from the small differences in the travel time of the sound from the birds to the different microphones, as well as from the differences in the loudness of the sound recorded at different microphones, we will be able to tell which song came from which bird.
Detection and identification of spikes of individual neurons are similar: the shape and amplitude of a neuron’s spike are slightly different when recorded from different distances relative to the neuron. Thus, in case of studies examining the activity of single neurons, recording devices with multiple electrodes are advantageous over single electrode devices since the additional information provided by multielectrodes may help to assign spikes to individual cells with a higher reliability. Single wire electrodes were therefore soon replaced by tetrodes, that is, neural probes with four closely-packed electrodes (usually four twisted wires). Tetrodes are still in use today since they are cheap and relatively easy to fabricate.
A few decades later, however, advancements in microelectromechanical systems (MEMS) and microfabrication technologies made it possible to create tiny devices in the micrometer regime with a high precision and great reproducibility. Neuroengineersexploited these technologies by fabricating thin neural probes containing a high number of small electrodes with the electrodes placed in various layouts on the implantable parts of these probes.
Usually, the base material of neural probes is silicon (thus they are called silicon probes) and they have needle-like, slender but rigid shafts with a width and thickness in the range of the thickness of a human hair (Figure 1.). In these silicon probes, the “wires” which record the brain’s electrical activity are usually made of a metal with good electrical properties (e.g. platinum, gold or titanium) and are sandwiched between layers of silicon oxide in the silicon needle where there is only a single small opening for each wire to contact the brain tissue. Since these wires still have a significant physical size (> 0.001 mm), the space occupied by them will be the primary constraint determining the width of the implantable silicon shaft. A wide shaft (> 0.2 mm) is not favorable for experimental use because it causes a higher damage to the brain tissue during insertion (e.g. rupturing blood vessels or killing neurons) than probes with a narrower shaft. Thus, the number of electrodes (wires) on a single shaft has basically an upper limit (~50-100) for these types of probes.
Fortunately, another technology (advanced lithography employed in back-end silicon CMOS (complementary metal–oxide–semiconductor) metallization) allows to pattern wires in the nanometer range and in multiple planes, therefore significantly increasing the number of electrodes that can be integrated on a probe. This enables neuroscientist to make silicon probes with a high number (> 100) of closely-packed electrodes which can sample the electrical activity of neurons with a very high spatial resolution (Figure 1 and 2).
In the framework of the successful EU-funded project NeuroSeeker (www.neuroseeker.eu), in collaboration with several European Research Institutes, Universities and a SME we have developed a high-density silicon probe containing 128 closely-packed, square-shaped electrodes arranged in a two-dimensional array (Figure 1; [1]). The interconnect routing was achieved using multi-layer CMOS back-end metallization. To illustrate how these probes record the neuronal activity, we can think of the electrode array as the image sensor of a CMOS camera (i.e. an array of pixels) and the recording of spikes as capturing a video of the neuronal activity. However, instead of recording only 24 images every second, the neural recording system maps the three-dimensional electric field produced by nearby neurons into a two-dimensional image containing 32 x 4 pixels twenty-thousand times per second.
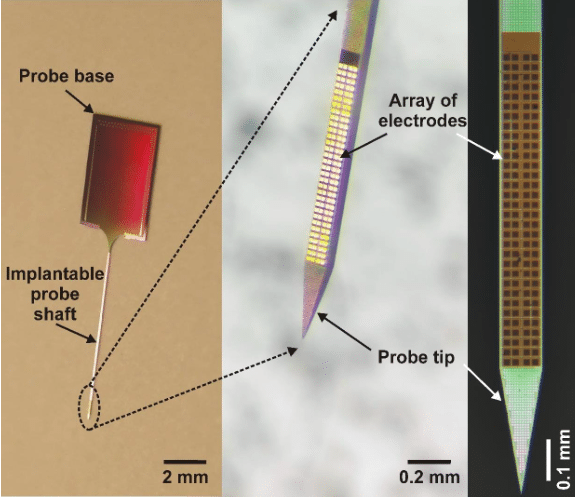
Figure 1. – The developed high-density silicon probe with 128 titanium nitride electrodes. The square-shaped electrodes are arranged in a 32 x 4 array. Credit: Richárd Fiáth
The developed high-density probe can record spikes in the brain of animal models (e.g. rats or mice) with a much higher spatial resolution as it is commonly done (Figure 2). The higher spatial resolution allows investigating neuronal spikes on a finer scale and with a higher spatial detail. This enables neuroscientists to examine the dynamics of action potential generation and propagation in living organisms.
Based on the densely sampled spike waveforms, researchers might also be able to differentiate between different types of neurons and examine their roles in brain computations. Furthermore, in the upcoming years, investigations carried out with these novel high-density probes may help to shed light on some of the mechanisms underlying various brain diseases like epilepsy, Alzheimer’s disease or Parkinson’s disease. That is why we are building a platform to exploit the technology and make it available for the international neuroscience community (for more information contact the project coordinator: Dr. Patrick Ruther, [email protected]).
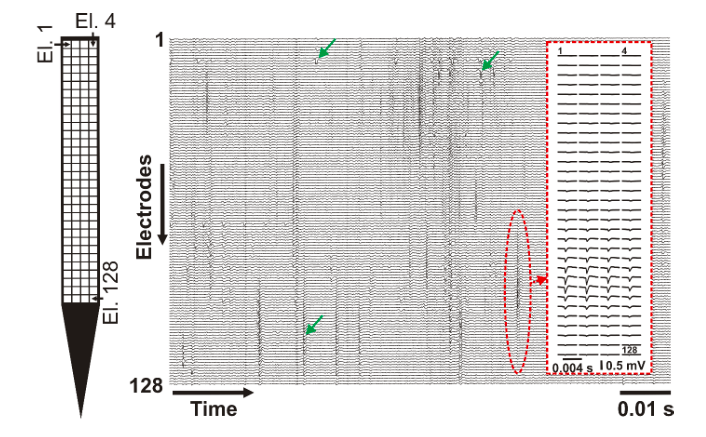
Figure 2. – A short (~0.1 s) recording showing spikes of multiple neurons obtained by the 128 electrodes. Example spikes are indicated with green arrows. The average spike waveform of an individual neuron is shown in the inset (red dashed rectangle). Left: the layout of the electrode array (El. – electrode). Credit: Richárd Fiáth
Neural probe technology continues to develop rapidly. Active CMOS probes with even higher numbers of electrodes were recently developed by the NeuroSeeker consortium [2] and also by other international research and development collaborations [3]. Besides electrodes, these state-of-the-art probes also contain active electronics on the silicon shaft, as well as on the probe base (similar to microchips). These integrated electronic components preprocess the incoming analog data flow (e.g. do the amplification or digitization of the signals) or can be used for example to select an arbitrary subset of electrodes for measurements.
These findings are described in the article entitled A silicon-based neural probe with densely-packed low-impedance titanium nitride microelectrodes for ultrahigh-resolution in vivo recordings, recently published in the journal Biosensors and Bioelectronics.
This work was conducted by Richárd Fiáth, Domonkos Horváth, and István Ulbert from the Research Centre for Natural Sciences of the Hungarian Academy of Sciences and Pázmány Péter Catholic University, Faculty of Information Technology and Bionics, Bogdan Cristian Raducanu and Chris van Hoof from the Interuniversity Microelectronics Center and KU Leuven, Silke Musa, Alexandru Andrei, and Carolina Mora Lopez from the Interuniversity Microelectronics Center, Patrick Ruther from the University of Freiburg, Department of Microsystems Engineering (IMTEK), and Arno Aarts from ATLAS Neuroengineering.
References:
- Fiáth R, Raducanu BC, Musa S, Andrei A, Lopez CM, van Hoof C, Ruther P, Aarts A, Horváth D, and Ulbert I. A silicon-based neural probe with densely-packed low-impedance titanium nitride microelectrodes for ultrahigh-resolution in vivo recordings. Biosens Bioelectron 106: 86-92, 2018.
- Raducanu BC, Yazicioglu RF, Lopez CM, Ballini M, Putzeys J, Wang SW, Andrei A, Rochus V, Welkenhuysen M, van Helleputte N, Musa S, Puers R, Kloosterman F, Van Hoof C, Fiáth R, Ulbert I, and Mitra S. Time Multiplexed Active Neural Probe with 1356 Parallel Recording Sites. Sensors 17: E2388, 2017.
- Jun JJ, Steinmetz NA, Siegle JH, Denman DJ, Bauza M, Barbarits B, Lee AK, Anastassiou CA, Andrei A, Aydin C, Barbic M, Blanche TJ, Bonin V, Couto J, Dutta B, Gratiy SL, Gutnisky DA, Hausser M, Karsh B, Ledochowitsch P, Lopez CM, Mitelut C, Musa S, Okun M, Pachitariu M, Putzeys J, Rich PD, Rossant C, Sun WL, Svoboda K, Carandini M, Harris KD, Koch C, O’Keefe J, and Harris TD. Fully integrated silicon probes for high-density recording of neural activity. Nature 551: 232-236, 2017.